To explore large-er aspect ratio wings; one fine morning, I just thought it would be fun to put a truss-braced wing in a Piaggio P.180 "Avanti". The modified design CAD files are is available here. A comparison is shown in Fig. 1. I am too lazy to make 2 separate airplanes so I modified half of it so I can run a CFD analysis using one model and one mesh 🤣. A slight modification about which I will write later is the positioning of the flaps and ailerons. These are moved to the truss part from the main wing in the original design. The aspect ratio is of the truss-braced section is double the original. With a foldable wing, storage shouldn't be a problem?
I write about Propulsion, Aerodynamics and Renewable Energy (Wind/Hydro Turbines).
Monday 17 April 2023
Executive transport aircraft with truss-braced wing (World's First)
Saturday 1 April 2023
Turbulent Fluid Structure Interaction (FSI) - Benchmark Case
After weeks spent self-learning about this type of simulation and countless nights spent troubleshooting this complex problem, I am pleased to share results. 😇 This post is about the FSI analysis of the FSI-PfS-2a. A case designed by Dr. Breuer. The geometry is shown in Fig. 1. The geometry details are available in ref. [1]. The geometry is made in SolidWorks CAD package and then imported to ANSYS via .STEP file. FSI combines Computational Fluid Dynamics (CFD) and structural analysis, i.e. the Finite Element Method (FEM).
References
[1] A. Kalmbach and M. Breuer, "Experimental PIV/V3Vmeasurementsofvortex-induced fluid–structure interaction in turbulent flow—A new benchmark FSI-PfS-2a", Journal of Fluids and Structures, Vol. 42, pp 369–387, 2013
Sunday 25 December 2022
Datacenter Visualization (Verified and Validated)
This simulation is done to create an aero-thermal digital twin of a datacenter using CFD. The details of datacenter are taken from [1]. The datacenter CAD model is shown in Fig. 1.
The simulation employs κ − ε turbulence model with damping functions, SIMPLE-R (modified), as the numerical algorithm and second-order upwind and central approximations as the spatial discretization schemes for the convective fluxes and diffusive terms. The time derivatives are approximated with an implicit first-order Euler scheme. Flow simulation solves the Navier–Stokes equations, which are formulations of mass, momentum, and energy conservation laws for fluid flows. To predict turbulent flows, the Favre-averaged Navier–Stokes equations are used.
A Cartesian mesh with octree refinement, cut-cell method and immersed boundary methods is used. Special mesh refinements are deployed in the areas of interest i.e. inlets and outlets and sharp edges of server racks and CRAH units to accurately capture aero-thermal gradients and vortices. The resulting computational mesh has 2,698,156 cells. The computational domain and mesh are shown in Fig. 2.
References
Saturday 10 September 2022
DARPA Suboff Submarine CFD Simulation (Backed-up by Water Tunnel Data); Update 01: Water Level Simulations (Volume of Fluid)
This post is about the CFD analysis of the DARPA Suboff at various speeds. The DARPA Suboff model is based on a generic submarine. The submarine geometry is shown in Fig. 1. The submarine in fully submerged in water. Refer to section "Update 01" for submarine sailing at the water level. The submarine geometry is available here. The geometry is made using equations from [1]. Machine Learning available here.
The simulations are validated with published literature [2-4]. SolidWorks Flow Simulation Premium software is employed for the simulations. Fig. 2 shows results of drag force at various cruise speeds. It can be seen that the results are in close agreement with the published experimental / numerical data.
The mesh has 656,714 cells in total. With 34,258 cells on the submarine surface. Special mesh refinements are added in the regions of interest i.e. regions with high gradients, the wake and on the control surfaces of the submarine. The mesh for is shown in Fig. 3.
Update 01
References
Tuesday 30 August 2022
SACCON CFD Simulation (Compared by Wind Tunnel Data)
This post is about the CFD analysis of the SACCON UCAV. Designed by NATO’s (North Atlantic Treaty Organization) RTO (Research and Technology Group) under Applied Vehicle Task Group (AVT-161) to assess the performance of military aircraft. The aircraft Geometry is shown in Fig. 1. The aircraft geometry is available here [1].
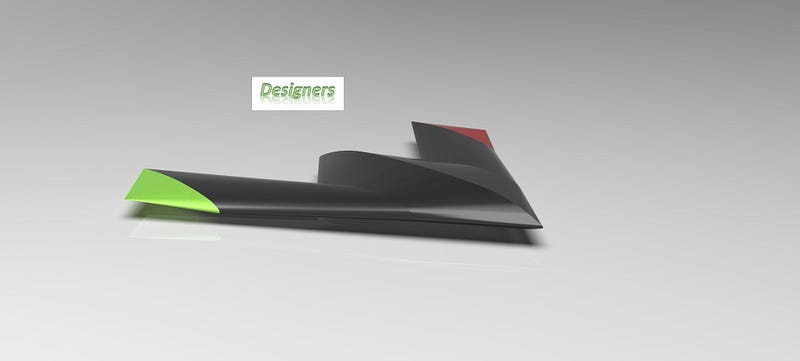
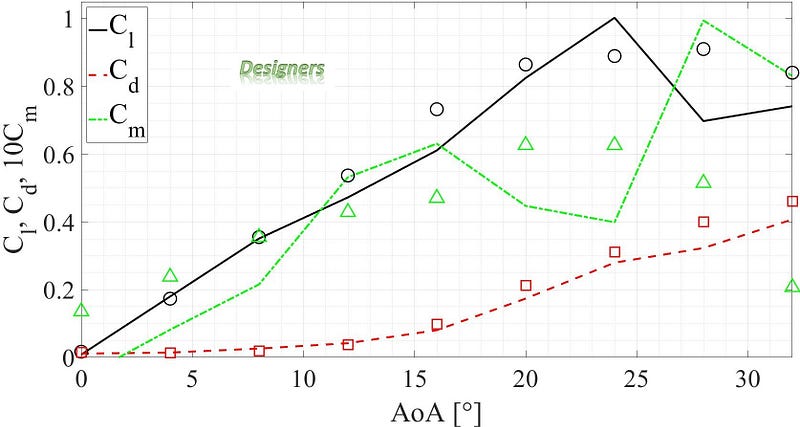
The mesh has 3.7 million cells in total. Special mesh refinements are added in the regions of interest i.e. regions with high gradients, the wake and on the control surfaces of the aircraft. The computational domain and the mesh for 16° angle of attack is shown in Fig. 3.
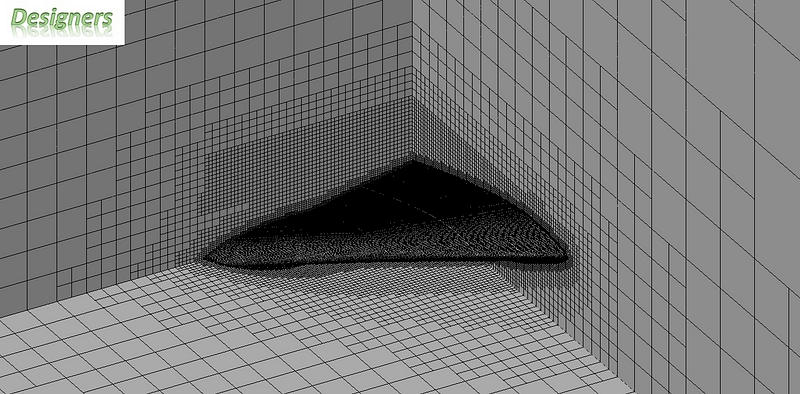
The mesh has 3.7 million cells in total. Special mesh refinements are added in the regions of interest i.e. regions with high gradients, the wake and on the control surfaces of the aircraft. The computational domain and the mesh for 16° angle of attack is shown in Fig. 3.
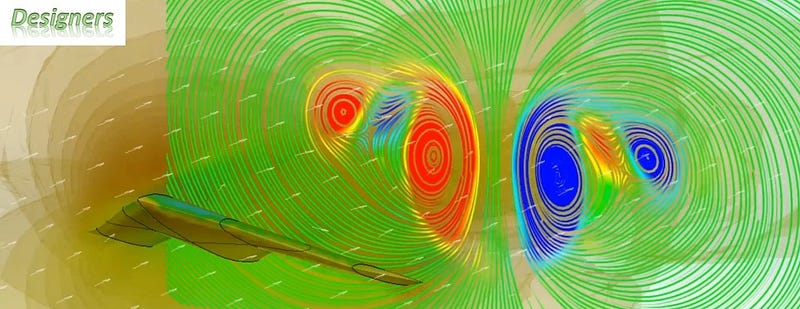
Thank you for reading, If you would like to collaborate on projects, please reach out.
References
[1] Andreas Schütte, Dietrich Hummel and Stephan M. Hitzel, “Flow Physics Analyses of a Generic Unmanned Combat Aerial Vehicle Configuration,” Journal of Aircraft, Vol. 49, No. 6, December 2012, https://doi.org/10.2514/1.C031386
Monday 18 July 2022
High Lift Common Research Model (CRM-HL) CFD Simulation (with validation from Stanford CTR and NASA) Update 01: Formation Flight
This post is about the CFD analysis of the nominal (2A) configuration of the Boeing / NASA CRM-HL with flap and slat angles at 40 and 37 degrees. The configuration is shown in Fig. 1.
The dimensions are mentioned in [1] and is available here and here. The numerical simulations are validated with published literature [3, 4]. SolidWorks Flow Simulation Premium software is employed for the CFD simulations. The flight conditions are Mach 0.2 at 289.444 K and 170093.66 Pa [2].
Fig. 2 shows the computational mesh along with the computational domain. The surface mesh is also shown with Fig. 2. It can be seen that the mesh is refined in the areas of interests and in the wake of the aircraft to properly capture the relevant flow features. The Cartesian mesh with immersed boundary method, cut-cell approach and octree refinement is used for creating the mesh. The mesh has 6.5 million cells, of which around 0.71 millions cells are at boundary of the aircraft.
The simulations employ κ-ε turbulence model with damping functions and two-scales wall functions. SIMPLE-R (modified) as the numerical algorithm. Second order upwind and central approximations as the spatial discretization schemes for the convective fluxes and diffusive terms. The software solves the Favre-averaged Navier-Stokes equations to predict turbulent flow. The simulations are performed to predict three-dimensional steady-state flow over the aircraft
Angle of attack of 7 and 17 degrees are considered. At 7 degree angle of attack, the drag life and moment coefficients are within 6, 8 and 12% of the published data, respectively. For 17 degree angle of attack, the coefficients are within 4, 7, 33% of the published data [3, 4]. On average, the results of the present simulations are within 6% of the published data for force coefficients and within 12% in terms of pitching moment coefficient. The pitching moment coefficient will improve with refinement of the mesh, as shown by [3]; which I will do if ever I convert this into a manuscript 😂. The post processing from CFD simulations is shown in Fig. 3-4. Within Fig. 3, the iso-surfaces represent vorticity in the direction of flow, colored by pressure. The direction of flow is shown by black arrows. Streamlines colored by vorticity are also visible. It can be seen from Fig. 2 that the simulation captures important flow features such as vortex formation very accurately, in such small number of mesh cells. Fig. 4 shows wing of the velocity iso-surfaces colored by vorticity in the direction of flow, focused around the wing.
The simulations are solved using 10 of 12 threads of a 4.0 GHz CPU with 32 GB of total system memory of which almost 30 GB remains in use while the simulations are in progress. To solve each angle of attack, 3 hours and 47 minutes are required.
Update 01
References
[1] Doug Lacy and Adam M. Clark, "Definition of Initial Landing and Takeoff Reference Configurations for the High Lift Common Research Model (CRM-HL)", AIAA Aviation Forum, AIAA 2020-2771, 2020 10.2514/6.2020-2771
[2] 4th AIAA CFD High Lift Prediction Workshop Official Test Cases, https://hiliftpw.larc.nasa.gov/Workshop4/OfficialTestCases-HiLiftPW-4-2021_v15.pdf
[3] K. Goc, S., T. Bose and P. Moin, "Large-eddy simulation of the NASA high-lift common research model", Center for Turbulence Research, Stanford University, Annual Research Briefs 2021
[4] 4TH High Lift Workshop Results, ADS, https://new.aerodynamic-solutions.com/news/18
Sunday 22 May 2022
Fifth Generation Fighter Aircraft CFD Simulation (Backed-up by Wind Tunnel Data)
This post is about the CFD analysis of a Sydney Standard Aerodynamic Model (SSAM-Gen5) in flight at various angles of attack. The SSAM-Gen5 model is based on the Lockheed Martin F-22 Raptor. The aircraft Geometry is shown in Fig. 1. The aircraft geometry is available here and here. Machine Learning can be read here.
Sunday 24 April 2022
1D Laplace's equation using Finite Difference Method
This post is about FDM for Laplace Equation with various boundary conditions.
MATLAB Code (1D, Dirichlet Boundary Conditions)
%% initialize the workspace, clear the command window
clear; clc
%% finite difference 1D laplace dirichlet boundary conditions %% d2u/dx2 = 0 %% u(o) = 10, u(L) = 4 %% Ax=b%%
N = 4 ; %number of grid points
L = 1; %length of domain
dx = L/(N-1); %element size
%% initialize variables %%
l = linspace(0,L,N); %independent
u=zeros(1,N); %dependent
%% boundary conditions %%
u(1)=10;
u(end)=4;
%% b vector %%
b=zeros(N-2,1);
b(1) = b(1) - u(1);
b(end) = b(end) - u(end);
%% A matrix
A = -2*eye(N-2,N-2);
for i=1:N-2
if i<N-2
A(i,i+1)=1;
end
if i>1
A(i,i-1)=1;
end
end
%% solve for unknowns %%
x = A\b;
%% fill the u vector with unknowns %%
u(2:end-1) = x;
%% plot the results %%
hold on; grid on; box on, grid minor
set(gca,'FontSize',40)
set(gca, 'FontName', 'Times New Roman')
ylabel('u','FontSize',44)
xlabel('l','FontSize',44)
plot(l,u,'-o','color',[0 0 0],'LineWidth',2,'MarkerSize',20)
MATLAB Code (1D, Mixed Boundary Conditions)
Wednesday 18 August 2021
Computational Fluid Dynamics Simulation of a Swimming Fish (Includes UDF)
This post is about the simulation of a swimming fish. The fish body is made of NACA 0020 and 0015 aero-foils (air-foils). The fluke is made of NACA 0025 aero-foil (air-foil), as shown in Fig. 1. the CAD files with computational domain modelled around the fish is available here.
Thursday 20 August 2020
The Fan Car
The idea to reduce Drag and/or improve Downforce on a vehicle using fans at the rear has been around for decades. Specially in the world of motorsports. Examples include Gordon Murray's BT46 and the T50. Here an explanation is made as to why placing a fan behind a car or a container-carrier truck can be used to improve fuel economy.
The sample car model is of the renowned Ahmed Body. For validation of the numerical simulation, please refer to this post.
Fig. 1 shows pressure isosurfaces around the car body both with and without fans installed at the rear. It is clear that the pressure difference between rear and front of the car is more when the fans are not available. More pressure difference results in more Drag and a relatively bad fuel economy.
Fig. 1, T-B; Fan disabled, fan enabled
Fig. 2 shows cross section view of the car. It can be seen that the the boundary layer is re-energized and as a result the flow separation is significantly reduced by adding a fan at the rear. By adding a fan, the vortices are not only moved away from the rear-end of the car but also have smaller size and less intensity, as shown in Fig. 3.
Fig. 2, T-B; Fan disabled, fan enabled. Red arrows represent direction of airflow
Fig. 3, L-R; Fan disabled, fan enabled
Thank you for reading. Please share my work. If you would like to collaborate on a project please reach out.
Saturday 8 August 2020
The Aerofoil
Fig. 3, Air flow is from left to right.
Fig. 5, Along the horizontal axis, 0 refers to leading edge.
Fig. 6, Air flow is from left to right.
Wednesday 15 July 2020
Aerofoil Kinematics Computational Fluid Dynamics (Update: 01)
Sunday 22 March 2020
Hypersonic Flow over a Two Dimensional Heated Cylinder
M Freestream Mach number at 17.6
Re Reynolds number at 376,000
μ Dynamic viscosity at 1.329045e-5 Ns.m-2
R Specific gas constant at 286.9 J.(kg.K)-1
T Freestream temperature 200 K
d Cylinder diameter at 5.6730225e-4 m
P Freestream pressure at 101325 Pa
γ Specific heat ratio at 1.4
Tw Wall temperature of cylinder at 500 K
Pr Prandtl number at 0.736